Bio-Integrated Electronics Explained: The Seamless Merger of Humans and Technology
Explore the fascinating world of bio-integrated electronics. Understand the core principles, materials, technologies, applications, and ethical considerations of merging biology with advanced electronics for healthcare and human augmentation.
Prerequisites
- Basic understanding of human biology (cell types, tissues, organs)
- Fundamental concepts of electronics (circuits, sensors, conductivity)
- Introductory knowledge of materials science (polymers, metals, semiconductors)
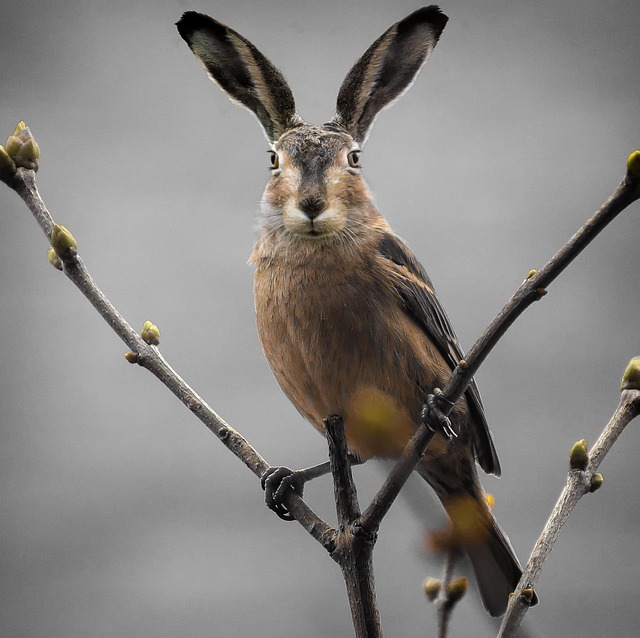
1. Introduction: Bridging the Biological and Electronic Worlds
The quest to understand and interact with the human body at its most fundamental levels has driven innovation for centuries. Bio-integrated electronics represent a paradigm shift in this endeavor, moving beyond externally worn gadgets or rigid, often isolated implants. At its core, bio-integrated electronics refers to a class of electronic devices specifically engineered to form a direct, intimate, and functional interface with biological systems - tissues, organs, and even individual cells. The goal is not merely co-location but a true physical and functional merger.
This field distinguishes itself sharply from conventional approaches. Traditional wearable sensors (like smartwatches) sit on the body's surface, separated by layers of air, clothing, and often rigid interfaces, limiting the quality and types of signals they can acquire. Conventional implants (like pacemakers or deep brain stimulators) are typically encased in rigid, hermetically sealed packages (often titanium) designed to isolate the electronics from the body's internal environment. While effective for specific functions, their rigidity creates a significant mechanical mismatch with the surrounding soft, dynamic biological tissues, potentially leading to inflammation, scarring (fibrosis), and device failure over time. Furthermore, their bulkiness limits where and how they can be deployed.
Bio-integrated electronics tackle this abiotic/biotic interface challenge head-on. The historical trajectory began with bulkier implants, gradually incorporating microfabrication techniques. However, the true revolution started with the realization that mimicking the mechanical properties of biological tissues - their softness, stretchability, and complex shapes - was crucial for seamless integration. Researchers like John Rogers and others pioneered approaches using novel materials and structural designs (like serpentine interconnects) to create electronics that could conformally laminate onto skin, wrap around organs, or interface with the brain's intricate folds.
The overarching vision is transformative. Imagine electronic systems that function as a natural extension of the body: skin-like patches continuously monitoring vital signs with clinical accuracy in a home setting; smart sutures detecting infection and releasing antibiotics precisely when needed; brain interfaces restoring communication for paralyzed individuals; or even systems that monitor organ health post-transplant and provide early rejection warnings. These systems aim to provide unprecedented access to biological information and enable targeted interventions.
Achieving this vision necessitates addressing several key goals: 1. Overcoming Mechanical Mismatch: Designing devices with effective moduli comparable to biological tissues (kPa to MPa range) to ensure conformal contact and minimize stress concentration. 2. Ensuring Biocompatibility: Selecting and engineering materials that do not elicit adverse immune responses, are non-toxic, and ideally promote integration with surrounding tissue. 3. Achieving Stable Signal Transduction: Ensuring reliable and high-fidelity transfer of signals (electrical, chemical, optical, thermal) across the electronic-biological interface over long periods, despite the challenging, dynamic physiological environment. 4. Developing Powering and Data Strategies: Creating sustainable power sources and wireless communication methods suitable for miniature, often implanted, devices.
This field represents a convergence of materials science, electrical engineering, biology, and medicine, pushing the boundaries of how technology can interface with life itself.
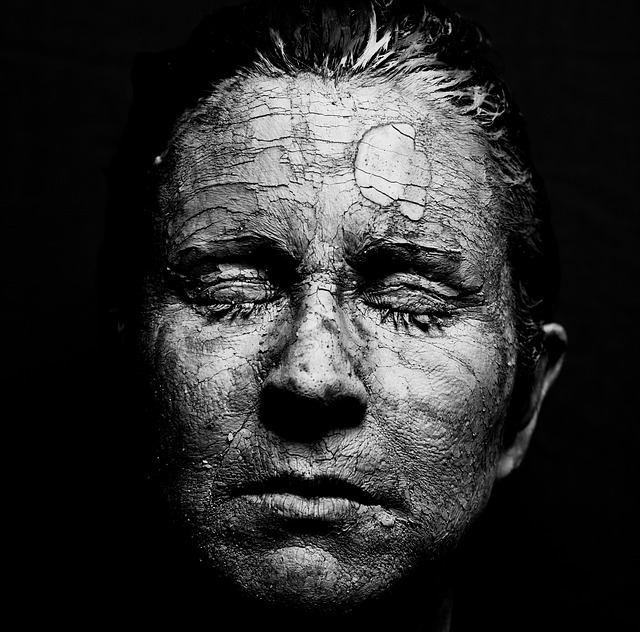
2. Core Principles and Enabling Materials
The successful integration of electronics with biological systems hinges on overcoming fundamental mismatches, primarily mechanical and biological. Traditional electronics, built on rigid silicon wafers, possess a Young's Modulus (a measure of stiffness) in the GigaPascal (GPa) range. In stark contrast, biological tissues like skin, muscle, and brain tissue exhibit moduli in the KiloPascal (kPa) to MegaPascal (MPa) range - orders of magnitude softer. This profound mechanical mismatch is a critical barrier. When a rigid device is placed in contact with soft tissue, especially under dynamic conditions (like breathing, muscle contraction, or simple movement), stress concentrates at the interface. This can lead to device delamination, inaccurate readings, tissue irritation, inflammation, and the formation of a stiff scar tissue capsule (foreign body response), which further isolates the device electrically and chemically.
Therefore, a core principle of bio-integrated electronics is to design devices with mechanical properties (softness, bendability, stretchability) that closely mimic those of the target biological tissue. This ensures conformal contact over complex, curvilinear surfaces and minimizes stress during movement, leading to more stable and reliable long-term interfaces.
Equally crucial is biocompatibility. This multifaceted concept refers to the ability of a material to perform its desired function without eliciting undesirable local or systemic effects in the host body. For bio-integrated electronics, this means materials must be non-toxic, non-immunogenic (or minimally so), and resistant to degradation modes that produce harmful byproducts. Depending on the application, materials might need to be biostable (resisting degradation for long-term use) or intentionally biodegradable (for transient devices that dissolve after their function is complete). Thorough biocompatibility testing (in vitro and in vivo) is essential for any material intended for biological interfacing.
These principles dictate stringent material requirements: * Softness/Low Modulus: Matching tissue stiffness. * Stretchability/Elasticity: Accommodating tissue deformation without failure. * Biocompatibility: Avoiding harmful biological responses. * Conductivity (Electronic/Ionic): Essential for sensors, actuators, and interconnects. Materials must often conduct electrons (like metals/semiconductors) and sometimes ions (like biological fluids). * Permeability: Allowing passage of water, ions, or biomolecules can be crucial for certain sensors or for reducing interfacial stress. * Biodegradability (Optional): Required for transient electronics.
Meeting these diverse requirements often necessitates the use of novel and composite materials: * Elastomers: Soft, stretchable polymers like Polydimethylsiloxane (PDMS) and Ecoflex serve as common substrates and encapsulation layers due to their low modulus, optical transparency, and established biocompatibility (though PDMS can adsorb proteins). * Hydrogels: Water-swollen polymer networks that closely mimic the hydrated environment and mechanical properties of soft tissues. They offer excellent biocompatibility and ionic conductivity but can be mechanically weak and challenging to integrate with dry electronics. * Conductive Polymers: Intrinsically conductive polymers (e.g., PEDOT:PSS) or polymer composites filled with conductive materials (e.g., silver nanoparticles/nanowires mixed into elastomers) provide stretchable conductivity. * Nanomaterials: Materials like carbon nanotubes (CNTs), graphene, and metallic nanowires (e.g., AgNWs, AuNWs) offer unique combinations of conductivity, mechanical strength, and flexibility. They can be incorporated into composites or used as thin films. * Thin-Film Metals/Oxides on Flexible Substrates: Traditional electronic materials (like gold, silicon nitride, indium tin oxide) deposited in ultra-thin layers (<100 nm) onto flexible or stretchable substrates. Often patterned into serpentine or buckled structures to accommodate strain.
Finally, the interfacial chemistry between the device surface and the biological environment is critical. Surface functionalization techniques can be employed to modify the device surface with specific molecules that promote cell adhesion, reduce protein fouling, improve electrical contact with neurons, or enhance biocompatibility. This chemical tailoring ensures the device not only sits passively but actively and appropriately interacts with its biological surroundings.
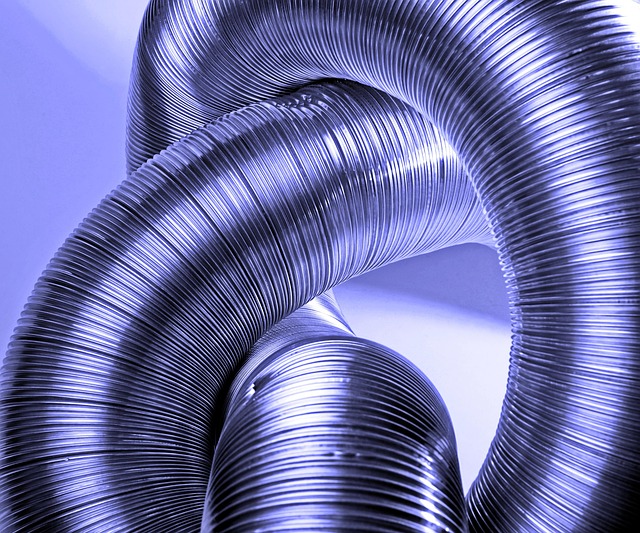
3. Key Technologies and Device Architectures
Leveraging the principles of mechanical compliance and biocompatibility, researchers have developed several key technology platforms within bio-integrated electronics, each tailored for specific applications and locations within the body.
Epidermal Electronics ('E-skin'): These are ultra-thin, lightweight, stretchable electronic systems designed to laminate conformally onto the skin's surface, much like a temporary tattoo. They adhere via van der Waals forces alone or with mild bio-adhesives, eliminating the need for straps or tapes. E-skin platforms can integrate a variety of sensors (temperature, strain, electrophysiological signals like ECG/EMG/EEG, hydration sensors, biochemical sensors analyzing sweat), actuators (haptic feedback elements, micro-heaters for transdermal drug delivery), wireless communication modules (NFC, Bluetooth), and power sources. Their non-invasive nature and ability to provide continuous, high-fidelity physiological data make them ideal for mobile health monitoring, sports performance tracking, and clinical diagnostics outside traditional settings.
Implantable Sensors and Actuators: Going beyond the skin, bio-integrated principles enable the design of soft, flexible devices for implantation within the body. These devices aim to monitor physiological parameters in deep tissue or specific organs, or to deliver therapeutic interventions with high precision. Examples include: flexible sensors to monitor intracranial pressure or temperature after brain injury; conformal electrode arrays wrapped around nerves or the spinal cord for recording neural activity or providing electrical stimulation for pain management or motor function restoration; soft, instrumented catheters providing real-time feedback during minimally invasive surgery; and implantable devices designed to monitor concentrations of specific biomarkers (e.g., glucose, lactate, pH) directly within interstitial fluid or blood. Actuation capabilities might involve integrated micro-pumps or reservoirs for highly localized, on-demand drug delivery triggered by sensor feedback (closed-loop systems).
Brain-Computer Interfaces (BCIs): A particularly compelling application area is interfacing directly with the brain. Traditional BCIs often use rigid penetrating electrodes (like Utah arrays) or low-resolution scalp EEG. Bio-integrated approaches offer significant advantages. Soft, flexible electrode arrays designed to conform to the brain's curved surface can record high-resolution Electrocorticography (ECoG) signals with greater stability and potentially less tissue damage than rigid implants. Even thinner and more flexible arrays, sometimes called Micro-ECoG (µECoG), can achieve spatial resolutions approaching those of penetrating electrodes while remaining on the cortical surface. These advanced BCIs hold promise for restoring communication and control for individuals with severe paralysis, understanding brain function, and potentially treating neurological disorders.
Transient Electronics: This fascinating sub-field involves creating high-performance electronic devices from bioresorbable materials - materials that completely and safely dissolve within the body over a predetermined period (days, weeks, or months). Components like conductors (e.g., magnesium, zinc, molybdenum), dielectrics (e.g., silicon oxides/nitrides, biodegradable polymers like PLLA/PLGA), and even silicon nanomembranes (as semiconductors) can be integrated onto biodegradable substrates. These devices can perform a specific function, such as monitoring infection post-surgery and releasing heat to kill bacteria, or providing temporary electrical stimulation to accelerate nerve regeneration, and then simply disappear, obviating the need for a second surgery for removal. This reduces patient risk, cost, and discomfort.
Powering Strategies: Providing power to these often miniaturized and sometimes implanted devices is a critical challenge. Several strategies are employed: * Wireless Power Transfer: Near-field communication (NFC) or inductive coupling allows power to be transferred wirelessly from an external source to the device, eliminating the need for implanted batteries for some applications. * Energy Harvesting: Converting ambient energy into electrical power. This includes piezoelectric or triboelectric generators harvesting mechanical energy from body movement (breathing, heartbeat), or biofuel cells generating electricity from biochemical reactions using substances like glucose and oxygen present in physiological fluids. * Thin-Film Batteries: Developing flexible, thin, and sometimes stretchable battery chemistries that can be integrated directly into the device architecture. Ensuring long-term stability and safety within the body remains an active area of research.
4. Fabrication and Integration Techniques
Creating sophisticated electronic devices that are also soft, stretchable, and biocompatible requires innovative fabrication and integration strategies that go beyond conventional semiconductor manufacturing.
Micro/Nanofabrication on Flexible Substrates: While standard photolithography, deposition, and etching processes were developed for rigid silicon wafers, they can be adapted for use with flexible polymer substrates like polyimide (PI) or parylene, or even temporary rigid handlers that are later removed. This allows for the creation of high-resolution electronic components (transistors, sensors, interconnects) using established, high-yield methods. However, challenges arise from substrate flexibility, thermal budget limitations of polymers, and managing stress in the deposited thin films.
Soft Lithography: This suite of techniques, pioneered by George Whitesides, utilizes patterned elastomeric stamps (typically made of PDMS) to pattern materials on various substrates, including flexible and curved ones. Key methods include: * Microcontact Printing (µCP): An elastomeric stamp inked with molecules (e.g., self-assembled monolayers, proteins) transfers the pattern onto a substrate upon contact. This is useful for chemical patterning or creating etch masks. * Replica Molding (REM): An elastomer prepolymer is cast against a master mold (often created by traditional lithography) and cured to replicate the features. This is fundamental for creating microfluidic channels or structured surfaces. Soft lithography is well-suited for patterning soft materials and biological molecules, offering lower cost and simpler infrastructure compared to traditional cleanroom processes, though often at lower resolution.
Additive Manufacturing (3D/4D Printing): 3D printing techniques, such as inkjet printing, direct ink writing (DIW), and stereolithography (SLA), are increasingly adapted for bio-integrated electronics. They allow for the layer-by-layer construction of complex, three-dimensional device architectures using a range of functional inks (conductive, insulating, stimuli-responsive, cell-laden). This enables rapid prototyping, customization for individual patients, and the integration of multiple functionalities within a single structure. 4D printing extends this by incorporating materials that can change shape or function over time in response to specific stimuli (e.g., temperature, pH, light), offering possibilities for self-folding or actuating devices after deployment.
Transfer Printing: Many high-performance electronic components (e.g., silicon nanomembrane transistors, LEDs) are best fabricated on their native rigid wafers but are needed on soft, stretchable substrates for bio-integration. Transfer printing is a crucial technique to bridge this gap. It involves selectively picking up these delicate micro/nano-scale components from the source wafer using a patterned elastomeric stamp and then printing them onto the target flexible/stretchable substrate in desired locations and arrangements. This allows the integration of high-performance, brittle materials into mechanically compliant systems.
Integration Strategies: Once fabricated, the device must be integrated with the biological system. This can occur via: * Surgical Implantation: For internal devices, requiring standard surgical procedures. * Minimally Invasive Delivery: Deploying devices through catheters or via injection, potentially using self-folding or injectable electronic designs. * Bio-adhesives: Using biocompatible glues or hydrogel adhesives for securing devices to tissues or skin. * Self-Assembly: Designing components that can spontaneously assemble into functional structures in situ (less common for full devices, more for molecular-scale interactions). * Conformal Lamination: For epidermal electronics, relying on van der Waals forces or gentle adhesion to follow skin topography.
Packaging and Encapsulation: Protecting the delicate electronic components from the harsh, wet, and corrosive biological environment is critical for long-term function. Traditional implants rely on bulky, rigid, hermetic sealing (e.g., titanium cans). For bio-integrated devices, which prioritize softness and conformability, this is often not feasible. Instead, thin-film encapsulation strategies using biocompatible barrier materials (like silicon dioxide, silicon nitride, parylene, or specialized polymers) are employed. Achieving perfect, pinhole-free conformal coatings that prevent ion and water penetration over long periods, while maintaining flexibility, remains a significant challenge and an active area of materials research.
5. Applications: Transforming Healthcare and Beyond
The unique ability of bio-integrated electronics to form stable, high-fidelity interfaces with biological systems opens up a vast landscape of potential applications, primarily focused on revolutionizing healthcare but also extending into fundamental research and even speculative future enhancements.
Continuous Health Monitoring: Perhaps the most immediate impact is in moving health monitoring from episodic clinical visits to continuous, real-time data acquisition in a person's natural environment. Epidermal electronics can track vital signs (ECG, respiration, temperature, blood oxygen saturation), activity levels, hydration status, and even stress indicators through sweat analysis. Implantable sensors could monitor intra-organ parameters like pressure (e.g., intracranial, intraocular, cardiovascular), temperature, or the concentration of specific metabolites (glucose for diabetes management, lactate for athletes or critically ill patients). This continuous stream of data provides a much richer, personalized picture of health, enabling early detection of deviations from baseline and facilitating proactive health management.
Advanced Diagnostics: By enabling sensitive detection of biomarkers directly within the body or in easily accessible biofluids like sweat or interstitial fluid, bio-integrated sensors promise earlier and more convenient disease diagnostics. This could involve detecting inflammatory markers for early signs of infection or implant rejection, specific proteins associated with cancer recurrence, or subtle changes in neurochemicals related to neurological disorders. The goal is to shift diagnosis from late-stage symptoms to early, subtle molecular or physiological signatures, enabling more effective and less invasive treatments.
Personalized Therapeutics: Bio-integrated electronics enable the development of closed-loop systems, where a sensor continuously monitors a physiological parameter, a processor analyzes the data, and an actuator delivers a therapeutic intervention automatically based on pre-defined algorithms. A classic example is the artificial pancreas, where a continuous glucose monitor informs an insulin pump to deliver appropriate doses. Bio-integrated approaches could refine this concept with fully implanted systems or enable similar closed-loop control for other conditions, such as automated drug delivery for pain management based on neural signals, or electrical stimulation adjusted in real-time based on therapeutic effect.
Neuroprosthetics and Rehabilitation: Interfacing directly with the nervous system offers profound possibilities for restoring lost function. Bio-integrated electrode arrays (surface or penetrating) can record neural signals associated with movement intention, allowing paralyzed individuals to control prosthetic limbs, computer cursors, or communication devices (BCIs). Conversely, electrical stimulation delivered through conformal electrode arrays can restore sensory input (e.g., cochlear implants, retinal prosthetics), alleviate chronic pain (spinal cord stimulation), manage symptoms of Parkinson's disease (deep brain stimulation), or promote nerve regeneration after injury.
Human Augmentation: While highly speculative and ethically charged, the potential exists for bio-integrated electronics to augment human capabilities beyond restoration or therapy. This could theoretically involve enhancing sensory perception (e.g., providing input for wavelengths of light or sound outside the normal human range), improving cognitive functions through direct neural stimulation or interfacing, or providing seamless access to information networks. These possibilities raise significant ethical questions but are part of the long-term vision explored by some researchers.
Research Tools: Beyond clinical applications, bio-integrated devices serve as powerful tools for fundamental biological research. They allow scientists to monitor complex physiological processes (e.g., neural signaling, tissue development, immune response) in vivo with unprecedented spatial and temporal resolution, providing insights that are difficult or impossible to obtain through traditional methods. Mapping brain activity during behavior, monitoring tissue oxygen levels during regeneration, or tracking cellular responses to stimuli in real-time are just a few examples.
6. Challenges and Future Directions
Despite the immense promise and rapid progress, the field of bio-integrated electronics faces significant hurdles that must be overcome to translate laboratory prototypes into widespread clinical and commercial realities. These challenges span materials science, engineering, data science, manufacturing, and regulation.
Long-Term Stability and Reliability: Perhaps the most critical challenge is ensuring devices function reliably and remain biocompatible for their intended lifespan, especially for chronic implants. The warm, wet, chemically active biological environment is harsh on electronic materials. Encapsulation layers must prevent moisture and ion ingress without failing due to mechanical fatigue or degradation. Material interfaces must remain stable. For electronics interfacing with dynamic tissues like the brain or heart, maintaining stable contact and signal quality over months or years despite micromotion and the body's foreign body response (fibrosis/scarring) is exceptionally difficult. Controlling the degradation rate precisely for transient electronics also remains challenging.
Power Management: Powering miniaturized, often implanted, devices remains a bottleneck. While wireless power transfer is viable for devices near the skin or those needing only intermittent power, it becomes inefficient for deep implants or continuous operation. Energy harvesting techniques currently provide very low power levels, often insufficient for complex computation or high-bandwidth wireless transmission. Thin-film batteries offer higher power density but face challenges related to longevity, safety (leakage, swelling), flexibility, and the need for recharging or replacement, which may require additional surgeries.
Data Management and Security: Bio-integrated systems, particularly for continuous monitoring or high-channel-count neural interfaces, can generate vast amounts of data. Transmitting this data wirelessly requires significant power and bandwidth, especially from within the body. On-device processing can reduce data load but requires more complex, power-hungry circuitry. Furthermore, ensuring the security and privacy of this highly sensitive physiological data is paramount. Robust encryption and secure communication protocols are essential to prevent unauthorized access or malicious manipulation, particularly for devices capable of actuation (e.g., drug delivery, stimulation).
Scalability and Cost-Effectiveness: Many current bio-integrated devices are fabricated using specialized, lab-scale techniques that are expensive and low-throughput. Transitioning to scalable manufacturing processes (e.g., roll-to-roll processing, high-volume additive manufacturing) while maintaining performance and reliability is crucial for broader adoption. The cost of novel materials and complex fabrication steps must be reduced to make these technologies accessible.
Regulatory Hurdles: As medical devices, particularly implants incorporating novel materials and functionalities, bio-integrated electronics face stringent regulatory scrutiny (e.g., from the FDA in the US or CE marking in Europe). Demonstrating long-term safety, efficacy, and biocompatibility through extensive pre-clinical and clinical trials is a lengthy and costly process. Clear regulatory pathways for these convergent technologies are still evolving.
Future Directions: Research continues to push the boundaries: * Multi-modal Sensing: Integrating sensors for multiple parameters (e.g., electrical, chemical, optical, thermal, mechanical) onto a single platform for a more holistic physiological assessment. * Advanced Closed-Loop Systems: Developing more sophisticated control algorithms, potentially incorporating artificial intelligence (AI) and machine learning (ML), to enable highly personalized and adaptive therapies. * Integration with AI/ML: Using AI/ML to decode complex biological signals (like neural data), analyze large datasets from continuous monitors to predict health events, and optimize therapeutic interventions. * Advanced Biomaterials: Developing new materials with improved biocompatibility, tailored degradation profiles, better electronic/ionic conductivity, self-healing capabilities, or intrinsic therapeutic properties. * Seamless System Integration: Improving wireless communication protocols, power harvesting efficiency, and data compression techniques for fully autonomous, unobtrusive operation.
7. Ethical Considerations and Societal Impact
The profound potential of bio-integrated electronics to monitor, diagnose, treat, and potentially augment human capabilities inevitably raises significant ethical and societal questions that must be addressed proactively alongside technological development.
Data Privacy and Security: These devices collect intimate, continuous physiological data. Who owns this data? Who controls access? How can it be protected from unauthorized access by employers, insurers, marketers, or malicious actors? Breaches could expose sensitive health information, leading to discrimination or misuse. For devices capable of stimulation or actuation, the security risks are even higher - unauthorized control could cause direct physical harm. Establishing robust data governance policies, strong encryption standards, and secure communication protocols is not just a technical challenge but an ethical imperative.
Autonomy and Consent: Obtaining truly informed consent is crucial, especially for implantable or cognitive-interfacing devices. Individuals must understand the device's capabilities, risks, limitations, data handling practices, and potential long-term consequences. For individuals with cognitive impairments or communication difficulties (who might benefit greatly from BCI technology), ensuring genuine consent and respecting autonomy poses complex challenges. Furthermore, how does continuous monitoring or closed-loop therapy affect an individual's sense of self-control and agency over their own body?
Equity and Access: As these advanced technologies develop, there's a risk of exacerbating existing health disparities. Will these devices be affordable and accessible to all who could benefit, or will they create a divide between those who can afford advanced monitoring, diagnostics, and potential augmentations, and those who cannot? Ensuring equitable access requires conscious effort in design, manufacturing, pricing, and healthcare system integration.
Safety and Potential Misuse: Beyond malfunction risks (e.g., material failure, battery issues), there's the potential for misuse. Could data be used coercively? Could BCIs be used for interrogation or manipulation? Could augmentation technologies be weaponized (dual-use concerns)? Thorough risk assessment must consider not only intended use but also potential unintended consequences and malicious applications.
Defining 'Normal' Human Function: Particularly concerning human augmentation applications, bio-integrated electronics force us to confront philosophical questions about what constitutes 'normal' human capability. If technologies allow for significantly enhanced senses, physical endurance, or cognitive abilities, how does this impact our definition of humanity, societal expectations, and potential pressures to 'upgrade'? Could this lead to new forms of social stratification or discrimination based on augmentation status?
Need for Robust Ethical Frameworks and Public Discourse: Addressing these complex issues requires more than just technical solutions. It necessitates the development of robust ethical frameworks, guidelines, and potentially regulations informed by ongoing dialogue involving researchers, clinicians, ethicists, policymakers, patients, and the public. Transparency about technological capabilities and limitations, open discussion about potential societal impacts, and careful consideration of values are essential to ensure that bio-integrated electronics are developed and deployed responsibly for the benefit of humanity.
The journey towards seamlessly merging humans and technology is as much an ethical and societal exploration as it is a scientific and engineering endeavor.
Further Reading
- Rogers Group - Northwestern University (Pioneering Research Lab)
- Some aspects of theory and fabrication for biointegrated electronics (Review Article)
- Epidermal electronics (Seminal Paper)
- Soft Microfluidic Assemblies of Sensors, Circuits, and Radios for the Skin (Research Article)
- Biointegrated and Bioresorbable Electronics for Healthcare (Review Article)